Black holes, as we understand them, come to us as predictions from Einstein’s general theory of relativity, which states that matter warps the fabric of spacetime.
Yes, we say. Of course.
I don’t really understand what this means, and so I look it up: general relativity for children. Everything about outer space makes me feel like a child.
Brittanica Kids tells me to imagine that spacetime is a flat rubber sheet, and that the Sun is a bowling ball. If you put the bowling ball on the rubber sheet, it will bend the rubber sheet around it. If you put a smaller ball, like a marble, next to the bowling ball, it will fall along the rubber sheet, toward the bowling ball.
Imagine that the rubber sheet encapsulates what Einstein called world-lines—the paths that all objects take as they move through spacetime. If you push the marble outward, it will travel along the trajectory promised by the push of your finger for a little bit—and then it will fall back toward the bowling ball, tracing an arc as it goes. This is the world-line of the marble. It is affected by the gravitational pull of the bowling ball, which warps the spacetime around it—the world-lines—and causes other, smaller objects with less mass to fall toward it.
This is different from Newtonian gravity, which argues that gravity is a singular force that acts equally upon all things. Both Newton’s theory of gravity and Einstein’s theory of relativity predict similar things when gravity is weak, but when gravity is strong—the closer you get to a star, for example—or when things are travelling at high speeds—the speed of light, for example—Newtonian calculations produce results that are off.
Take Mercury, for example. By the early twentieth century, three hundred years of planetary observations had shown astronomers that the planet’s orbit moved in a precession, a slow rotation of the orbit over time, so that each time the planet returned to the same place on its elliptical orbit it was actually shifting by a number of degrees.
Newton’s law of gravity, accounting for the pull of the Sun and the other neighbouring planets as forces that also pulled on Mercury’s orbit, calculated that the planet’s precession should develop by 5,557 inches every century, but actual measurements showed that the precession rate was 5,600 inches.
Einstein’s theory, postulating warping in the fabric of spacetime due to Mercury’s proximity to the Sun (here the Sun is a bowling ball, and Mercury the marble), calculated the 5,600 inches perfectly.
Matter warps the fabric of space and time.
The more matter you have, the greater the warping.
If enough matter gathers, the warping will be so great that even light cannot escape it.
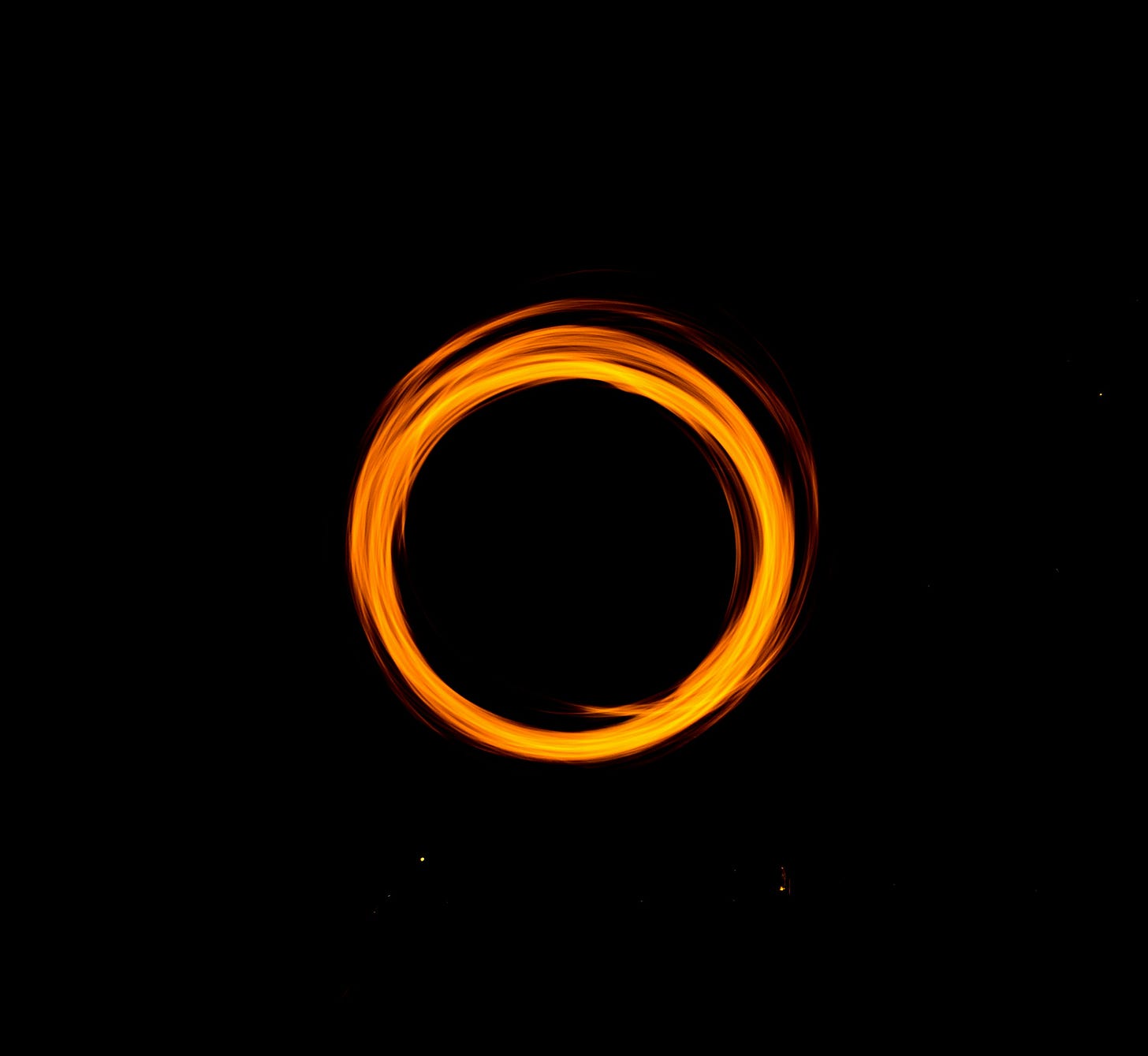
In her book Black Hole Survival Guide, astrophysicist Janna Levin says it like this: “[A] black hole is a place, a place in space and time…better: a black hole is spacetime.”
An emptiness from which nothing escapes.
But also not an emptiness, because it is densely full, densely packed with all of the matter inside of it that has also ceased to be.
In the immediate days after Jess died, I walked around unstitched by the immensity of the black hole that had suddenly appeared. It was everywhere—in front of me, inside my ribcage, everywhere I looked. That vast emptiness where my soulmate had once stood, packed simultaneously with nothing and also with the everything that she had been.
You could live until you’re one hundred, I kept saying to myself that first week. Over and over.
That first week, the next one, the next one after that.
I still say it now, and Jess died over four years ago.
You could live until you’re one hundred.
And if you do, you’ll have to live all that time without her.
Before we get much farther, and lest you think that I know what I am talking about, let me assure you: I do not. I can read about the history of these experiments and get familiar with the kid-friendly versions of Einstein’s work, but I don’t really understand what the numbers mean.
Matter warping the fabric of spacetime? Okay.
Accumulations of matter that are so dense they go beyond warping and create anomalies in spacetime? Okay. I’ll take your word for it.
What I’m most interested in is Einstein himself, not believing. Einstein himself, arguing against the existence of black holes. In a 1939 paper published in the Annals of Mathematics, he concluded that the presence of a conglomeration of matter so dense that not even light could escape it was “not convincing”, and “didn’t exist in the real world”. But in the end, it was his own theory that proved the conglomeration’s very existence.
In 2015, gravitational waves—ripples in the fabric of spacetime created by the merging of two distant black holes, exactly as predicted by Einstein’s math—were observed by the LIGO (Laser Interferometer Gravitational-Wave Observatory, comprising two locations—one in Hanford Site, Washington, the other in Livingston, Louisiana) for the very first time.
The first photographic evidence of a black hole wouldn’t meet the world until a few years after this, in April of 2019, over a century after Einstein’s theory first predicted their existence.
It took over a hundred years, but we got there.
April of 2019, several days after Jess turned thirty-seven. April of 2019, almost eight months before she died.
Before, I think. And after.
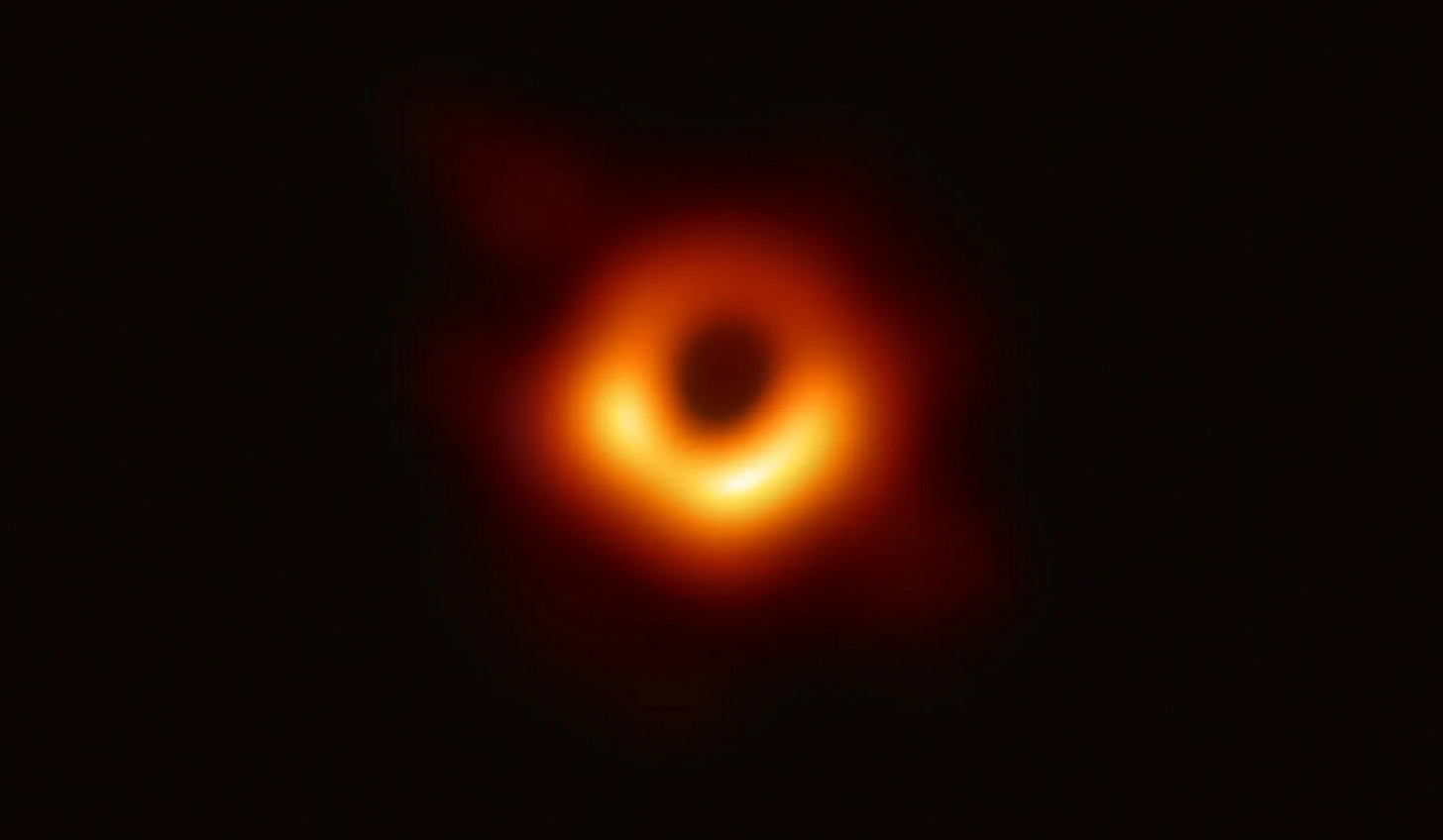
Years after Jess dies, I watch a video of Janna Levin holding a yellow tennis ball. “I could crush this in my hand until it’s very small,” she says. She’s speaking to a child who is maybe nine years old. “It would weigh the same, but it’s much more dense.”
The title of the video is Astrophysicist Explains Black Holes in 5 Levels of Difficulty. The five levels of difficulty: child, teen, college student, grad student, expert.
The point: we think of black holes as massive because they are so dense, but they’re actually quite small, especially when measured against the vastness of everything around them. A black hole with the mass of our Sun would have an event horizon only six kilometres across.
When the teen sits down in front of her, Janna says it like this: Imagine that you’re plucking the strings on an electric guitar. You can’t hear the sound of the strings until the amplifier is plugged in. In the same way, the gravitational waves of a black hole can’t be heard or measured unless we have the right amplifier—like the LIGO—to reveal them.
The LIGO allows us to capture gravitational waves floating out there in space and then reconstruct them—to isolate the frequency and the pitch of the waves the same way that an amplifier plugged into a guitar allows us to determine other things—the note, the length of the string played, how hard the string was plucked.
We get the data and work backwards. When we get the data from the amplifier, we can reassemble the guitar. When we get the data from the LIGO, we can reassemble the timeline back two distant black holes, merging into one another. This frequency, this pitch, this vibration in the wave—all of these things translate to distance and time.
But the gravitational waves can only take us back so far. They take us back to the black hole, but no farther.
What went into the black hole, we wonder? What kinds of stars, what kinds of planets? An errant spaceship that strayed too close?
We don’t know. We can’t measure. Light itself cannot escape the gravity of a black hole, cannot go into the black hole and then fight its way out. And light is the fastest thing in our universe.
If light can’t do it, then there isn’t yet a way to send someone into the black hole, and back out to us, to report on their findings.
When the college student sits in from of her, Janna leans out of concrete metaphor and farther into the not-knowing. “The beauty of being a student of something like black holes,” she says, “is that you never stop having new impressions of what this enigmatic phenomena is.”
The college student, Jayda, has pink hair and glasses and seems like someone I would be friends with. Janna Levin does too, if I’m honest, though she seems much smarter than I am and thus that much more terrifying.
Still, I can’t help watching her and imagining what she might say if I was to sit across from her in that chair.
What does a black hole feel like?
Does it feel like love? Does it feel like what happens when your best friend and soulmate dies and you walk around feeling so hollow you’re not sure you even have a heart anymore?
I don’t know, I imagine Janna saying. But really—who knows? In a year, I’ll tell you what I’ve learned that’s new.
Something I often forget: we call it Einstein’s theory of spacetime.
The physical data—certainly from black holes—bears it out. But it is still a theory, in the same way that Ptolemy’s geocentric theory was the prevailing approach to the universe for over 1,500 years.
Theoretical astrophysicists. We understand. But also we do not understand, at the same time. We understand only until something else comes to change the picture, to trade one theory for another.
Black holes come about as a result of stars that collapse under their own weight, and maybe as a result of other things we don’t yet understand. Their mass is so great that they warp the fabric of spacetime. Nothing can escape them, not even light. We cannot retrieve the matter that falls into a black hole and reconstitute its parts to determine its history.
So far, so good.
But the astrophysicist Stephen Hawking, world-famous thanks to his work on black holes, theorized that black holes do, in fact, let something back out into the vast bowl of space. This something is called Hawking radiation.
Hawking’s theory states that electromagnetic energy must be released by the outer layer of the black hole’s event horizon—that point of no return from which nothing is supposed to escape. Electromagnetic energy must be released, in turn, because everything in the universe that has a temperature emits this kind of energy. The outer layer of a black hole, made up as it is of stars funnelling into the abyss, is very hot.
Ergo: black holes must emit radiation. And if they emit radiation, then they can slowly dissipate over time. Billions and billions of years.
So far, so good, again.
But Hawking radiation is thermal, which can only carry information about the temperature of the object emitting it. It is thermal because as the stars get siphoned away into the black hole, their particles split and separate. The particle of a particle pair goes into the abyss. The anti-particle of a particle pair comes back out as thermal radiation. And thermal radiation can’t carry information—molecules that can be reconstructed, electrons and photons that can be modelled back to a previous state of being—in the way that electromagnetic radiation does. So if information isn’t escaping the black hole, but the presence of Hawking radiation means that the black hole is still slowly being siphoned away into nothing over billions of years, where does the information go?
Quantum mechanics tells us that information cannot be destroyed. You cannot, actually, have something that then becomes nothing. The information is still there, somewhere.
We just have to learn how to read it.
In a 2022 article published in the journal Physical Review Letters, physicists Xavier Calmet, Roberto Casadio, Stephen D. H. Hsu, and Folkert Kuipers argued that black holes do emit radiation that can carry information. They did this by factoring quantum gravity into Hawking’s equations, effectively showing that the gravitational field around a black hole contains a “fingerprint” of the matter that went into the hole. The radiation emitted is, therefore, not thermal, and can contain information on the matter that went before it.
This is just a theory too. Maybe, we say. Maybe, maybe.
We don’t have instruments sophisticated enough to measure this just yet. Perhaps one day we will. Perhaps, someday far into the future, our telescopes will bring back data that can tell us what black holes really are, and where they go. What happens to all of that nothing from something.
You never stop having new impressions.
After Jayda the college student, Janna sits across from Clare, a graduate student in astrophysics. Clare is studying star formation histories in the Small and Large Magellanic Clouds, which lie roughly 200,000 and 160,000 light-years away from us.
Janna looks at Clare head-on. “Does the Large Magellanic Cloud have a big black hole?”
“So,” and Clare tilts her head as though she’s confessing a secret, “I think the prevailing wisdom for a while was no, but my answer—honestly?—is that I’m not sure.”
“Probably nobody is,” Janna says, and both women laugh.
Clare’s not sure, I think. She’s a graduate student in astrophysics and she’s not sure. I find this impossibly comforting.
You never stop having new impressions, Janna reminds me.
The science and the math tell us that black holes form primarily from stars that collapse under their own weight. But the stars need to be heavy enough for this to happen—otherwise, they’ll balloon out into cooler red giants before shrinking into white dwarf stars.
This issue here, as with so many other things about space, has to do with time. It takes time for stars to be born and die and explode and get collected into the debris that swirls into other stars, heavier stars, which then collapse into black holes. A truly massive black hole, if it is formed from a star’s collapse, needs billions and billions of years to accomplish this. Many more billions of years than the 13.7 billion years that comprise our universe.
So if we suspect that there are massive black holes at the centre of so many of the universe’s galaxies, but the math doesn’t bear out the time needed for these black holes to form through star collapse alone, how do they come to be?
Here’s a wrench in the machine: the larger a black hole is, the less dense the material that makes it has to be.
“It might be,” Janna says to Clare, “that in the very early universe, bubbles in unusual high energy phase transitions—from a high energy universe to a low energy universe—could make black holes. We haven’t really thought of the range of possibilities.”
As I listen to her, it feels somewhat like standing on the edge of a black hole of my own. Small and tiny in the face of something so vast and huge. All of the things that we don’t know aggregated into a gaping black void, visible only because of the whisper of things that we do recognize—stars, planets, wispy clouds of galaxies—swirling around it.
But what are these things that we recognize, anyway? What are these things that we think we understand?
I understand so little about all of these things. But I keep coming back to the images, the possibilities, the metaphors. A massive star collapses under its own weight, becomes a black hole, pulls new things into orbit and reshapes the entire fabric of space around its absence, or around its being—the density of what it has become, the utter weight of all of that nothing.
Isn’t this, too, the process of grieving? Something that was is now winked out of existence. You can’t reach into the nothing to retrieve it. You can’t call it back. Instead you swirl around that great soft hole of nothing-and-something, the space in front of you lit up with memory and light.
Quasars, which are formed from the galactic cores of stars speeding into the vortex of black holes, are some of the brightest objects in our universe.
There is something here, and I keep circling it the way that all of that dust and debris and gas and light swirls around the black hole, slowly dissolving into nothing (or into something, just a something we can’t yet read).
The Andromeda Galaxy, our nearest galactic neighbour, sits two-and-a-half million light years away from where we sit in the Milky Way. Observations of the galaxy over the years have shown us that Andromeda also has a massive black hole at its centre. It is also moving toward us, and we are moving toward it. Four billion years from now, give or take, our galaxies will collide.
But our galaxies are so large, and the space between our stars so astronomical, that in many cases—though not all—the collision of our galaxies will be more like a drift. Stars sweeping past one another, oblivious to all of the change that’s going on.
Our black holes will eventually merge. This process will take millions of years. And because the math tells us that the combined mass of black holes remains the same after a merger—leaving aside, for the moment, the infinitesimal leaking of Hawking radiation—the eventual black hole that will rest at the centre of the newly-formed Milkomeda (or Andromeway? Which one do you like better?) Galaxy will be very, very, very big. So massive—and still relatively so small—as to defy comprehension.
Some stars will collide with others, yes. Galactic collisions and chaos galore. Humans will likely have disappeared from our part of the universe long before this; whoever weathers the collisions in our place will have to ride out whatever happens in the wake of these unimaginable things.
But some planets—some species, some civilizations—will continue on as normal, with only a faint twinge of the change that is remaking the universe above their skies.
In the wake of the destruction? More gas, more clouds, more stars, and then—long billions of years later, perhaps—more planets. The combined galaxy will become something entirely new, set on an altogether different trajectory.
“[Black holes]”, says Clare the graduate astrophysicist, “are not just life-takers. They’re life-givers. And they inform a lot about how a galaxy is not just destroyed or made, but how it’s shaped, and how it builds life, like ours.”
The final person that Janna Levin speaks to in her video, the expert, is named Dan. He likes the information paradox—that conundrum set up by Hawking radiation.
What he really likes about the information paradox is the fact that Hawking had to draw quantum mechanics into the fray.
“A way to think about Hawking radiation,” he says, “is to imagine that pairs of particles and anti-particles appear out of the quantum vacuum. And the particle can escape the black hole, but the anti-particle falls in. But the particle and the anti-particle are a pair. And if the anti-particle really falls in the black hole and is destroyed in the singularity, that poor particle outside the black hole has lost his partner. [This] violates the rules of quantum mechanics.”
He’s talking about entanglement theory, which basically states that once two particles—a particle and an anti-particle, for example—become entangled with one another, they will always be entangled, even if they are then separated.
One-half of an entangled particle pair goes up, and the other half will go up. One-half of an entangled particle goes down, and the other half goes down. Even if it’s light-years away.
I don’t understand this at all. But neither did Einstein, which makes me feel much better.
Einstein called entanglement spooky action at a distance.
But neither Dan nor Janna seem particularly freaked out.
“We know more and more,” Janna says in the final voiceover for the video, “but we also see how much more there is to understand.”
Imagine: one-half of an entangled friendship dies, goes into the black hole, disappears.
What happens to the other half?
What wisdom do you have for the left-alone anti-particle, quantum mechanics, as it spins there in the universe, seemingly alone?
My favourite theory about black holes is more pseudo than science. This theory builds on the idea that in addition to black holes, the mathematics of gravity also predicts what is known as a white hole—an object (a place, says Janna Levin) with an event horizon just like a black hole, but with a singularity that spews matter out into the universe, faster than the speed of light.
When you couple a black hole with a white hole, you get what is known as an Einstein-Rosen bridge.
When you couple an Einstein-Rosen bridge with imagination, you get a wormhole. A portal from here to who-knows-where and who-knows-what.
The math tells us that if white holes exist (and most scientists think that they don’t, that they still reside firmly in the area of theory), they’ll probably be very unstable. You wouldn’t be able to send a single electron into a white hole without it collapsing.
But you wouldn’t be able to send an electron into a white hole anyway. You’d send the electron into the black hole, and gravity would suck the electron into the singularity at the black hole’s centre. And then you’d hope very hard that the electron makes it across and finds itself spewed out the white hole, into whatever awaits. You’d never be sure either way.
Imagine, though. A black hole, a white hole. An infinitely dense point from which everything, instead of disappearing, explodes into being.
What if something else is there? What if something other than nothing waits on the other side of that velvet blackness?
What if, from the complete end of one universe and everything you know, something else comes into being?
What happens then?
Currently Reading: The Art of Solitude, by Stephen Batchelor
Currently Watching: The Expanse (slowly, expansively)
Currently Eating: homemade rhubarb and raspberry crisp, courtesy of last year’s rhubarb from the garden (have to clear out the freezer to make room for more!)
Currently Substacking: The Feral Stack, by Summer Koester
Wow !! What an absolutely amazing read ! I find your Sunday Letters so interesting Amanda. I went out to look at the stars tonight. I find comfort, and , the more I read , a whole new understanding of how insignificant we really are on this small planet in this vast universe of ours. Thank you !